Rhône AGG Description/Documentation
Chapter 2: The Rhône
Database |
The Rhône is the largest European river which
empties into the Mediterranean Sea. The corresponding basin covers a
significant region of south-eastern France, encompassing a large
range in climatic conditions (continental, Mediterranean and Alpine
climates) and surface characteristics (bare mountains, forest, crop
land, etc.). An outline of the Rhône basin model domain is shown
relative to France and western Europe in Fig.
2.1. Surface characteristics, sub-surface parameters and
atmospheric forcing have been mapped onto this domain for use in
atmospheric and hydrological model studies. The atmospheric forcing,
the surface vegetation and soil parameter database, and the river
discharge and snow depth observation data are described in detail in
Habets et al. (1999b), Etchevers et al. (2001), Golaz et al. (2001) and Ottlé et al. (2001).
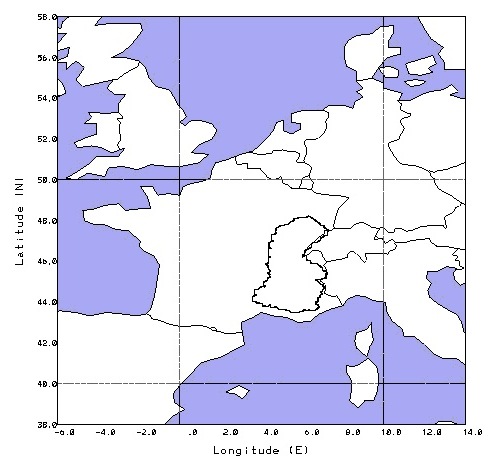 FIG. 2.1 The Rhône basin model
domain (highlighted area).
Atmospheric Forcing
The domain is divided up into 1471 8 km x 8 km
grid boxes for the atmospheric forcing (Fig.
2.2). The forcing is calculated using the SAFRAN (Systême
d'Analyse Fournissant des Reseignements Atmosphérique a la Neige)
analysis system (Durand et al.
1993), which was designed to compensate for the large variation
in elevation in the Rhône Alps (0-4800 m). The topography used for
the 8x8 km grid is shown in Fig. 2.3. The
atmospheric data utilized by the system consist of standard screen
level observations at approximately 60 Météo-France weather network
sites within the domain, ECMWF analysis and climatological data. In
addition, total daily precipitation data from over 1500 gauges are
incorporated into the system. The basin is divided into 249
homogeneous climatic zones which have a unique set of climatological
parameters. The locations of the climatic zones (outlined), rain
gauges (crosses) and meteorological stations (filled triangles) are
shown in Fig. 2.4.
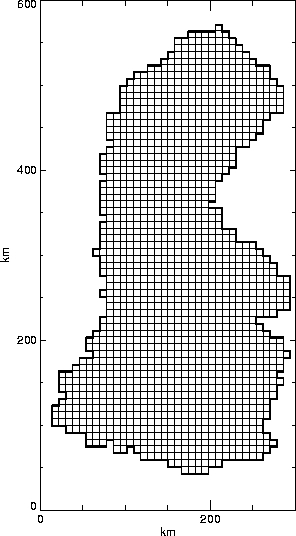
FIG. 2.2 The meteorological grid used for the SVAT scheme. There
are 1471 8 km x 8 km grid boxes within the Rhône
domain.
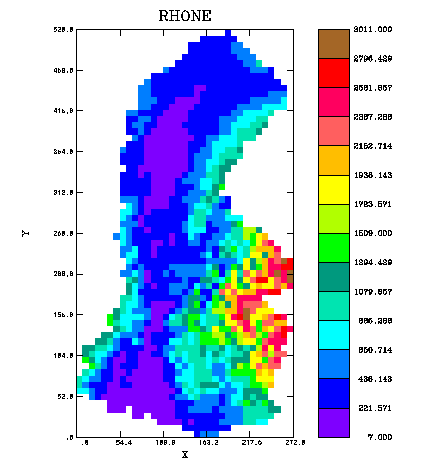 FIG. 2.3 The 8x8 km topography
for the Rhône basin model domain.
SAFRAN computes the vertical profile of each
of the atmospheric variables every six hours within each climatic
zone. The parameters are then interpolated to one hour intervals and
to the grid. The total daily precipitation is analyzed using the
observational data together with a vertical gradient of
precipitation (with altitude) derived from climatology. The
precipitation is then distributed hourly following the daily cycle
of observed humidity and the weather type (again from climatology).
Precipitation is partitioned between rainfall and snowfall based on
the 0.5 C isotherm (see Habets et al.
1999b for more details).
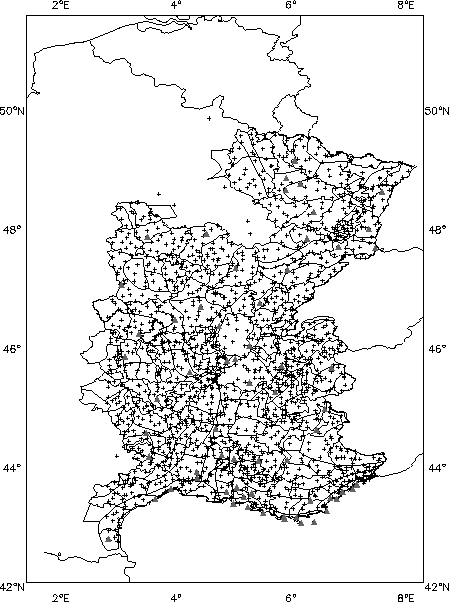 FIG. 2.4 The
locations of the climatic zones (outlined), daily rain gauges
(crosses) and meteorological stations (red triangles) used by SAFRAN
to derive the atmospheric forcing.
Downwelling radiative fluxes are calculated
using the radiative transfer scheme of Ritter and Geleyn (1992) together with
ECMWF and cloudiness analyses. The forcing extracted from SAFRAN for
the Soil Vegetation Atmosphere Transfer (SVAT) model computational
grid is available at a three hour time step. The provided forcing
variables are; air temperature at 2 m (Ta: K),
wind speed at 10 m (Va: m s-1),
specific humidity at 2 m (qa: kg kg-1),
down-welling visible solar radiation (Rg: W
m-2), down-welling long-wave atmospheric radiation
(Rat: W m-2), liquid precipitation rate
(Pl: kg m-2 s-1) and liquid
water equivalent snow/solid precipitation rate
(Ps: kg m-2 s-1). The
surface pressure (p: Pa) only varies as a function of
altitude, but the input values are provided at the same time step as
the other forcings (for ease of usage by the models). Problems
relating to model partitioning of precipitation into snow and rain
are avoided as the distinct components are provided. The forcing
variable definitions, sign conventions and units follow the ALMA land-surface data
convention.
Forcing is available for 17 years (1981-1998),
however, only four years will be used in the current study (August
1, 1985-July 31, 1989). These particular years were selected in
order to be consistent with the GSWP (Dirmeyer et al. 1999) database. The
starting and ending times are during the summertime due to the
importance of a good initialization of the snow cover in the
mountainous regions.
The 4-year average liquid and solid
precipitation components are shown in Fig.
2.5, and the 5 other 4-year average forcing variables (together
with the total precipitation) at each grid point are shown in Fig. 2.6. The monthly domain-average forcing
variables for the period August 1, 1985, through July 31, 1989, are
shown in Fig 2.7. The winter seasons in
1985-1986 and 1986-1987 were two of the three coldest in the forcing
dataset for the period 1981-1998 (the other exceptionally cold
winter was in 1984-1985).
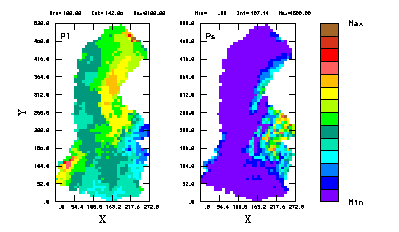 FIG 2.5 The 4-year average
precipitation forcing variables from the Rhône database from August
1, 1985 to July 31, 1989: Pl (liquid: 100-2100
with an interval of 143) and Ps (solid: 0-1500
with an interval of 107) in kg m-2.
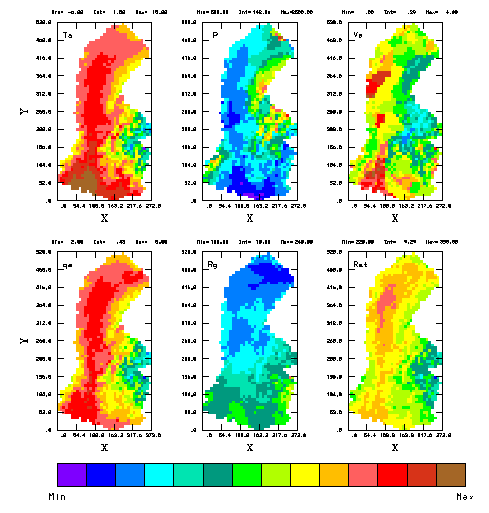 FIG 2.6 The 4-year
average forcing variables from the Rhône database from August 1,
1985 to July 31, 1989: Ta (C: -6 - 15 with an
interval of 1.5), P (kg m-2: 500-2500 with an
interval of 143), Va (m s-1: 0-4 with
an interval of 0.29), qa (g kg-1: 2-8
with an interval of 0.43), Rg (W m-2:
100-240 with an interval of 10) and Rat (W
m-2: 220-350 with an interval of 9.3).
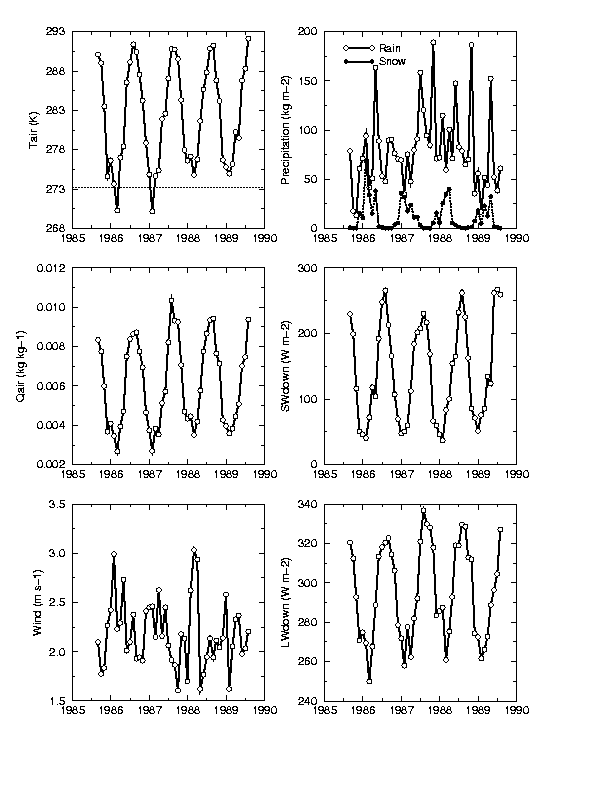 FIG 2.7 The 4-year
domain-average monthly mean forcing variables from the Rhône
database from August 1, 1985 to July 31, 1989.
Soil and Vegetation Parameters
Soil and vegetation parameter data are
available at the same spatial resolution as the atmospheric forcing.
The soil parameters are defined using the soil textural properties
(sand and clay fractions) from the INRA soil database (King et al. 1995). The spatial
distributions of the sand and clay fractions are shown in Fig.s 2.8a and 2.8b,
respectively.
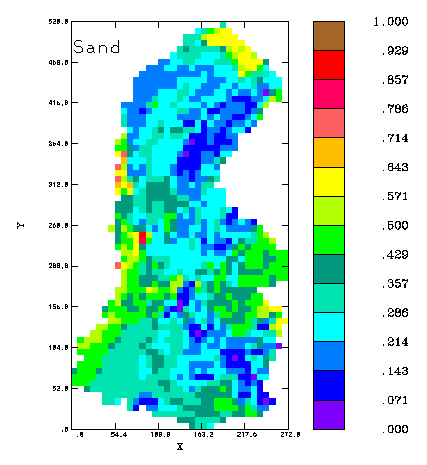 FIG. 2.8 The soil sand fraction
for each grid box.
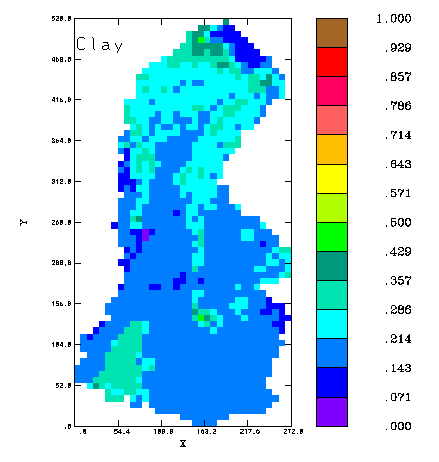 FIG. 2.8 The soil clay fraction
for each grid box.
The vegetation parameters are defined using a
vegetation map from the Corine Land cover Archive (Giordano et al. 1990) and a two-year
satellite archive of the AVHRR/NDVI Index (Champeaux and Legléau, 1995). There
are 10 distinct surface types considered, and the relative
fractional coverage of each surface type within each grid box was
used to determine average values for the 8 km x 8 km grid boxes. The
10 dominant surface types are listed with their corresponding index
in Table 2.1. The dominant surfaces are
shown in Fig. 2.9; bare rock (9), deciduous
forest (10), coniferous forest (8), grassland (7), cereal crops (3
and 5), crops other than cereals (1, 2 and 4), and mixed grassland
and crops (6).
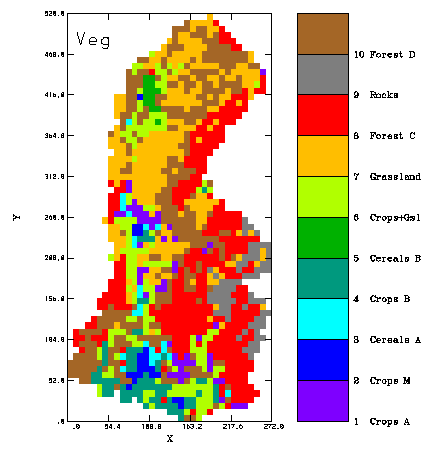 FIG 2.9 The dominant land
classification for each grid cell; 1) crops type A, 2) Mediterranean
crops, 3) cereals type A, 4) crops type B, 5) cereals type B, 6)
crops and grassland, 7) grassland, 8) coniferous forest, 9)
rocks/bare soil and 10) deciduous forest.
Maximum and minimum vegetation parameter
values are determined for each class. These values are then used
with the monthly observed NDVI to determine the evolution of the
roughness length, vegetation cover fraction and Leaf Area Index
(LAI). Similar surface types (such as Cereals A and B) can have
different temporal evolutions due to differences in the observed
NDVI. The single annual cycle for these parameters is to be applied
to all years simulated by the participant models. See Habets et al. (1999b) and Etchevers et al. (2001) for more
details.
TABLE 2.1 The ten surface types specified within the Rhône
model domain.
Class |
Description |
1 |
Crops A |
2 |
Mediterranean Crops |
3 |
Cereals A |
4 |
Crops B |
5 |
Cereals B |
6 |
Crops and Grassland |
7 |
Grassland |
8 |
Coniferous Forest |
9 |
Rocks |
10 |
Deciduous Forest
|
Distributed hydrological model data
Surface and sub-surface hydrological grids
have also been defined for use by distributed hydrological models.
Topographical data is used to determine the surface hydrographic
network and the water transfer time constant between each
hydrological grid cell (Golaz 1999).
The maximum transfer time for water to reach the outlet of the basin
was calibrated using the MODCOU model together with the observed
streamflow data.
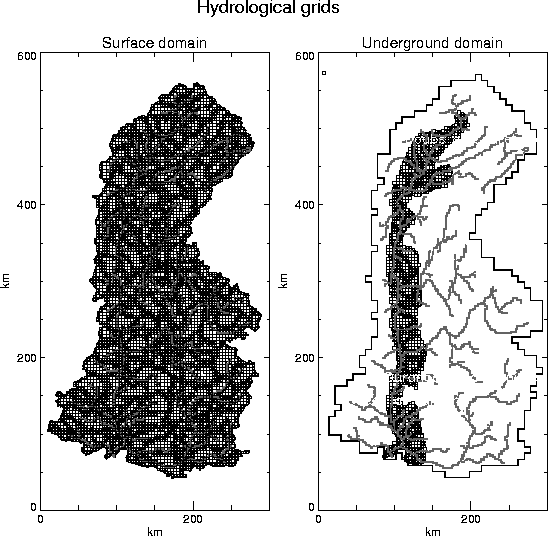
FIG 2.10
Surface and underground MODCOU grids.
The evolution of the water table is a function
of transmissivity and storage coefficients which have also been
calibrated using the observed streamflow. MODCOU uses a two-layer
approach, and the grid networks are shown in Fig.
2.10. The underground layer encompasses the aquifers for the
Rhône and Saone valleys and is approximately 21 % of the total
surface area of the basin, while the surface layer corresponds to
the atmospheric forcing domain and contains 27054 grid
elements.
Validation Data
There are two sets of observations which are
utilized for validating model simulations for the Rhône basin. The
first consists of 24 daily snow depth observation sites located
within the French Alps, which is used to evaluate the snow depth
prediction and timing of Spring snow melt simulated by the snow
cover module. The locations of the sites are shown in Fig. 2.11. A general evaluation will be done as
the depth measurements correspond to a point or a field, and the
simulated snowpack will correspond to the average value over an 8 km
x 8 km grid box. The obvious drawback of using depth measurements is
that model biases in simulated snow density can impact this
variable. From a hydrological standpoint, the snow water equivalent
(SWE) would form the basis for a more consistent inter-comparison
between the models and with the observations, however, SWE was not
observed. The snow depth is still useful, however, as it can be used
to infer the timing of the snowpack ablation.
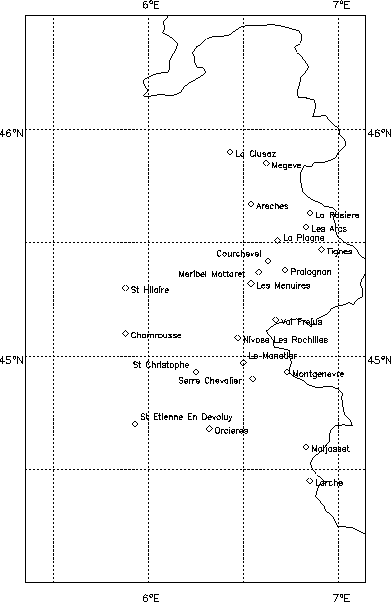
FIG. 2.11
Stations at which daily snow depth measurements are available.
The second set of observations consist of 145
river gauges which are used for validation of the simulated
streamflow. The location of the gauges for the 10 largest basins are
shown in the left panel of Fig. 2.12. The
relationship between the rivers and the relief is shown in the right
panel. For more information, see Habets et al. (1999b) and Etchevers et al.
(2001).
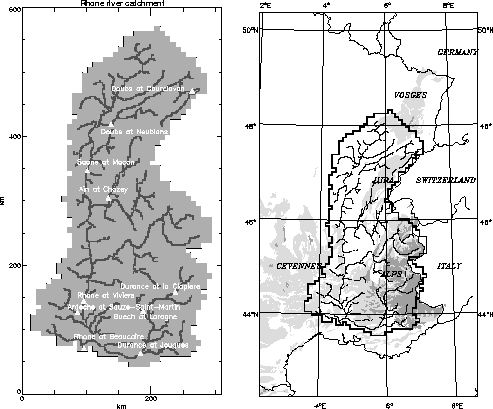
FIG. 2.12 Locations of
the gauging stations for the 10 largest sub-basins within the Rhône
basin are shown in the left panel. The relationship between the
major rivers and the relief is shown in the right panel (from Habets et al. (1999b)).
|